Research on the Dysbiosis in Dogs: Research and Hope for New Treatments
June 2, 2021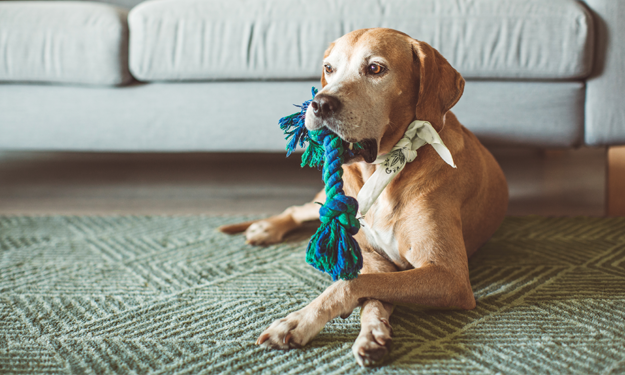
The gut microbiome, a collection of trillions of microorganisms living in the intestines, plays an essential role in regulating health and preventing disease. Ethos Discovery is investigating dysbiosis in dogs, the metabolic function of the microbiome and its impact on the body, moving beyond simply identifying the bacteria present to understanding how they work. In this blog you will learn:
- About the role the gut microbiome plays in not just digestion, but our overall health.
- About metabolites—such as short-chain fatty acids and secondary bile acids—that gut bacteria produce and how they influence processes like inflammation, immune regulation, and metabolism.
- How Ethos Discovery is using metabolomics to uncover how gut bacteria contribute to chronic diseases, and develop new diagnostics and treatments for both dogs and humans.
Introduction
The intestinal microbiota (bacteria, fungi) are highly functional, with a combined genome (termed the microbiome) that is approximately 130-160 times larger than the canine genome. The microbiota of the large intestine (and to a lesser extent the small intestine) are collectively becoming recognized as a metabolically active organ that affects mammalian physiology and health. There are up to 1014 microorganisms (predominantly anaerobic bacteria) belonging to more than 1,000 different species, in the colon. The majority of these bacteria belong to the phyla Firmicutes and Bacteroidetes, with the third and fourth most prominent being the phyla Actinobacteria and Proteobacteria. The collective microbiota contributes hundreds of thousands of additional genes to its host organism. This in turn has a tremendous impact on the number and diversity of metabolic reactions that can occur within the host. As the microbiota research field moves from initial, descriptive studies to more functional and mechanistic analyses, metabolomics is emerging as an important technology to enable discoveries about the function of the microbiome. In other words, analyzing and understanding the function of this group of organisms is much more important than characterizing which bacteria are present or absent. Currently available fecal testing is limited because it does not account for bacteria that are present in other areas of the GI tract. More importantly, fecal testing to analyze the inhabitants does not help us to understand the function of the microbiota.
What is Metabolomics?
Metabolomics is the scientific study of chemical processes involving metabolites, the small molecule substrates, intermediates, and products of cell metabolism. Specifically, metabolomics is the “systematic study of the unique chemical fingerprints that specific cellular processes leave behind”, the study of their small-molecule metabolite profiles. Metabolomics is the identification and quantification of small molecules that can provide a snapshot of the functional state of a cell/tissue. Metabolites produced by the intestinal microbiota, for example, are important modulators of host physiology. As we probe deeper into the canine and human microbiome, a core microbial population remains elusive, but metabolomics data are beginning to define a core function profile that may one day be diagnostic for homeostasis or dysbiosis.
Metabolic Function of the GI Microbiome
The metabolic function of the GI microbiome is just beginning to be studied and discovered. There are already some well-known contributions from the microbiota to mammalian health and physiology, and I will describe a few examples in this section.
Fatty acids: short chain & medium chain
Short chain fatty acids (SFCAs) are some of the most important metabolites produced by the gut microbiome. Dietary fibers resist digestion and absorption in the GI tract and can only be fermented by commensal bacteria in the gut, such as certain species from the Bacteroidetes and Firmicutes phyla. When gut bacteria break down fiber, they primarily produce short-chain fatty acids such as acetate, propionate and butyrate1. Scientists are discovering these short-chain fatty acids are incredibly important for our health because they are involved in several bodily functions2,3 such as:
- maintaining glucose stability
- regulating appetite
- providing fuel for intestinal cells
- maintaining the intestinal cell barrier
- regulating the immune system
- reducing inflammation
- regulating host gene expression
- cancer inhibition4
Secondary bile acids
Bile acids (BAs) are highly effective detergents that facilitate the hydrolysis and absorption of dietary lipids. Primary BAs, cholic acid (CA), and chenodeoxycholic acid (CDCA), are produced in the liver, and may be transformed by intestinal microbiota to secondary BAs such as deoxycholic acid (DCA) and ursodeoxycholic acid. One important discovery in the last 2 decades is that BAs are also signaling molecules that regulate several metabolic pathways including glucose utilization, fatty acid synthesis and oxidation, mitochondrial uncoupling, and BA production and transportation. Secondary bile acids have a number of other beneficial effects, including protecting against intestinal inflammation, production of beneficial effects in cases of primary biliary cirrhosis (PBC), primary sclerosing cholangitis, gallstones, digestive tract diseases, cystic fibrosis, and cancer. Ursodeoxycholic acid (UDCA) was the only drug approved by the US Food and Drug Administration (FDA) for the treatment of PBC. In addition, BAs have hypoglycemic properties and can aid in the treatment of Diabetes mellitus.7
Indolepropionic acid
3-indolepropionic acid (IPA) is a strong antioxidant produced by some gut bacteria that can help protect the nervous system from damage8. Research has shown low levels of IPA may play a role in the development of type 2 diabetes9,10 and research in animal models suggests IPA may also be involved in maintaining the gut barrier11. IPA is formed by breaking down the amino acid tryptophan.
There are many other metabolites produced by the GI microbiome, including phenolic acids, many essential vitamins, polyamines, and the aromatic amino acids tyrosine, phenylalanine and tryptophan. With the initial use of global metabolomics profiling, we will be able to uncover differences between groups of dogs in order to pinpoint a metabolite or group of metabolites that may be important in the development of a particular disease process.
Microbiome Function Contributing to Development of Disease
Example: The metabolite TMAO and its role in cardiovascular disease, impaired renal function, and colorectal cancer 12
A major role played by the intestinal microbiota is to aid in the harvest of nutrients from the diet13. Dietary components that are not readily absorbed in the small intestine serve as growth substrates for members of the gut microbiota, which in turn can modify the bioavailability and nutritional properties of those same dietary components13. For example, many of the beneficial effects associated with consumption of whole grains, vegetables, and fruits are at least in part mediated by end products of microbial metabolism, including short-chain fatty acids (e.g., butyrate) and phenolic acids (e.g., protocatechuic acid)14-18. Likewise, gut microbes can also convert otherwise beneficial dietary compounds, such as choline, into metabolites that are detrimental to human health19-22. Choline is required for a wide range of biological activities, including maintaining the structural integrity of cell membranes, supporting cholinergic neurotransmission, and donating methyl groups in a number of biosynthetic reactions23. Although choline is synthesized endogenously, this synthesis does not meet the levels necessary for optimal health23. Previous studies have established that gut microbial metabolism of choline results in the production of trimethylamine (TMA)24-26. Once TMA is absorbed by the host, it is further metabolized by flavin monooxygenases 1 and 3 (FMO1 and FMO3) in the host liver to generate trimethylamine-N-oxide (TMAO)20, 27, 28. Recent human studies have established that the levels of TMAO in serum are positively correlated with impaired renal function, colorectal cancer, and cardiovascular disease (CVD)20, 22, 29, 30. TMAO exacerbates atherosclerosis in a genetic knockout mouse model, in part by promoting forward cholesterol transport and by inhibiting reverse cholesterol transport20, 22, 31, 32. In addition, TMAO exacerbates impaired glucose tolerance, obstructs hepatic insulin signaling, and promotes adipose tissue inflammation of mice maintained on a high-fat high-sugar diet33. Future studies aimed at understanding how to manipulate the representation of choline consuming TMA-producing bacteria in the gut microbiota or at identifying species that modulate host conversion of TMA to TMAO might lead to novel interventions for preventing or treating atherosclerosis and/or choline deficiency-associated diseases12.
Systemic Consequences of Intestinal Dysbiosis
The impact of alterations in the gut microbiome on gut and systemic health are the topic of extensive new research. These systemic consequences may include immune-mediated disease, cancer, impaired wound healing, asthma, endocrine dysfunction, atopy, and epilepsy. Unfortunately, current approaches to measure the dysregulation of the intestinal microbiome exclusively assess the alteration in the gut microbiome but do not address the need, which is to alert clinicians to alterations in the gut microbiome that may impact systemic health, in order to prioritize interventions to correct these alterations. The unanswered question that needs to be answered: Are there metabolites that are produced in the gut with dysbiosis that can be measured in the blood and associated with specific systemic consequences of gut dysbiosis?
Ethos Discovery Dysbiosis Program: Metabolomics Study Future Goals
Through our work in the Ethos Discovery Dysbiosis Program, we endeavor to discover metabolites that are produced by the GI microbiome in dogs that have significant effects on systemic diseases. Our ultimate goal is to discover and develop novel diagnostics and therapeutics for intestinal dysbiosis, and ultimately to be able to predict and even prevent certain chronic diseases. This will lead to prolonged lifespan and health-span for domestic animals and also for human beings.
References:
1) den Besten, G. et al. The role of short-chain fatty acids in the interplay between diet, gut microbiota, and host energy metabolism. J. Lipid Res. 54, 2325–2340 (2013)
2) Morrison, D. J. & Preston, T. Formation of short chain fatty acids by the gut microbiota and their impact on human metabolism. Gut Microbes 7, 189–200 (2016)
3) Koh, A., De Vadder, F., Kovatcheva-Datchary, P. & Bäckhed, F. From Dietary Fiber to Host Physiology: Short-Chain Fatty Acids as Key Bacterial Metabolites. Cell 165, 1332–1345 (2016)
4) Microbiome and metabolome in diagnosis, therapy, and other strategic application. Faintuch 2019.
5) Lajczak-McGinley NK, Porru E, Fallon CM, Smyth J, Curley C, McCarron PA, Tambuwala MM, Roda A, Keely SJ. The secondary bile acids, ursodeoxycholic acid and lithocholic acid, protect against intestinal inflammation by inhibition of epithelial apoptosis. Physiol Rep. 2020 Jun;8(12).
6) Sidhartha R. Sinha, Yeneneh Haileselassie, Linh P. Nguyen, Carolina Tropini, Min Wang, Laren S. Becker, Davis Sim, Karolin Jarr, Estelle T. Spear, Gulshan Singh, Hong Namkoong, Kyle Bittinger, Michael A. Fischbach, Justin L. Sonnenburg, Aida Habtezion, Dysbiosis-Induced Secondary Bile Acid Deficiency Promotes Intestinal Inflammation, Cell Host & Microbe, Volume 27, Issue 4, 2020, Pages 659-670
7) Šarenac, T.M., Mikov, M., 2018. Bile Acid Synthesis: From Nature to the Chemical Modification and Synthesis and Their Applications as Drugs and Nutrients. Frontiers in Pharmacology 9.
8) Bendheim, P. E. et al. Development of indole-3-propionic acid (OXIGONTM) for alzheimer’s disease. J. Mol. Neurosci. 19, 213–217 (2002).
9) de Mello, V. D. et al. Indolepropionic acid and novel lipid metabolites are associated with a lower risk of type 2 diabetes in the Finnish Diabetes Prevention Study. Sci. Rep. 7, 46337 (2017).
10) Tuomainen, M. et al. Associations of serum indolepropionic acid, a gut microbiota metabolite, with type 2 diabetes and low-grade inflammation in high-risk individuals.
Nutr. Diabetes 8, 35 (2018).
11) Venkatesh, M. et al. Symbiotic Bacterial Metabolites Regulate Gastrointestinal Barrier Function via the Xenobiotic Sensor PXR and Toll-like Receptor 4. Immunity 41, 296–310 (2014).
12) Romano KA, Vivas EI, Amador-Noguez D, Rey FE. Intestinal Microbiota Composition Modulates Choline Bioavailability from Diet and Accumulation of the Proatherogenic Metabolite Trimethylamine-N-Oxide. mBio. 2015;6(2):e02481-14.
13) Tremaroli V, Bäckhed F. 2012. Functional interactions between the gut microbiota and host metabolism. Nature 489:242–249. http://dx.doi.org/ 10.1038/nature11552.
14) Xu J, Bjursell MK, Himrod J, Deng S, Carmichael LK, Chiang HC, Hooper LV, Gordon JI. 2003. A genomic view of the human-Bacteroides thetaiotaomicron symbiosis. Science 299:2074–2076. http://dx.doi.org/ 10.1126/science.1080029.
15) Martens EC, Lowe EC, Chiang H, Pudlo NA, Wu M, McNulty NP, Abbott DW, Henrissat B, Gilbert HJ, Bolam DN, Gordon JI. 2011. Recognition and degradation of plant cell wall polysaccharides by two human gut symbionts. PLoS Biol 9:e1001221. http://dx.doi.org/10.1371/ journal.pbio.1001221.
16) Donohoe DR, Garge N, Zhang X, Sun W, O’Connell TM, Bunger MK, Bultman SJ. 2011. The microbiome and butyrate regulate energy metabolism and autophagy in the mammalian colon. Cell Metab 13:517–526. http://dx.doi.org/10.1016/j.cmet.2011.02.018.
17) Bäckhed F, Manchester JK, Semenkovich CF, Gordon JI. 2007. Mechanisms underlying the resistance to diet-induced obesity in germ-free mice. Proc Natl Acad SciUSA 104:979–984. http://dx.doi.org/10.1073/ pnas.0605374104.
18) Vitaglione P, Donnarumma G, Napolitano A, Galvano F, Gallo A, Scalfi L, Fogliano V. 2007. Protocatechuic acid is the major human metabolite of cyanidin-glucosides. J Nutr 137:2043–2048.
19) Zeisel SH, daCosta KA, Youssef M, Hensey S. 1989. Conversion of dietary choline to trimethylamine and dimethylamine in rats: doseresponse relationship. J Nutr 119:800–804.
20) Wang Z, Klipfell E, Bennett BJ, Koeth R, Levison BS, DuGar B, Feldstein AE, Britt EB, Fu X, Chung Y-M, Wu Y, Schauer P, Smith JD, Allayee H, Tang WHW, DiDonato JA, Lusis AJ, Hazen SL. 2011. Gut flora metabolism of phosphatidylcholine promotes cardiovascular disease. Nature 472:57–63. http://dx.doi.org/10.1038/nature09922.
21) Tang WHW, Wang Z, Levison BS, Koeth RA, Britt EB, Fu X, Wu Y, Hazen SL. 2013. Intestinal microbial metabolism of phosphatidylcholine and cardiovascular risk. N Engl J Med 368:1575–1584. http://dx.doi.org/ 10.1056/NEJMoa1109400.
22) Koeth RA, Wang Z, Levison BS, Buffa JA, Koeth RA, Wang Z, Levison BS, Buffa JA, Org E, Sheehy BT, Britt EB, Fu X, Wu Y, Li L, Smith JD, DiDonato JA, Chen J, Li H, Wu GD, Lewis JD, Warrier M, Brown JM, Krauss RM, Tang WHW, Bushman FD, Lusis AJ, Hazen SL. 2013. Intestinal microbiota metabolism of L-carnitine, a nutrient in red meat, promotes atherosclerosis. Nat Med 19:576 –585. http://dx.doi.org/ 10.1038/nm.3145.
23) Zeisel SH, da Costa K-A. 2009. Choline: an essential nutrient for public health. Nutr Rev 67:615–623. http://dx.doi.org/10.1111/j.1753 -4887.2009.00246.x.
24) Zeisel SH, Wishnok JS, Blusztajn JK. 1983. Formation of methylamines from ingested choline and lecithin. J Pharmacol Exp Ther 225:320–324.
25) De la Huerga J, Popper H. 1951. Urinary excretion of choline metabolites following choline administration in normals and patients with hepatobilary disease. J Clin Invest 30:463– 470. http://dx.doi.org/10.1172/ JCI102463.
26) Hayward HR. 1960. Anaerobic degradation of choline. III. Acetaldehyde as an intermediate in the fermentation of choline by extracts of Vibrio cholinicus. J Biol Chem 235:3592–3596.
27) Shih DM, Wang Z, Lee R, Meng Y, Che N, Charugundla S, Qi H, Wu J, Pan C, Brown JM, Vallim T, Bennett BJ, Graham M, Hazen SL, Lusis AJ. 2015. Flavin containing monooxygenase 3 exerts broad effects on glucose and lipid metabolism and atherosclerosis. J Lipid Res 56:22–37. http://dx.doi.org/10.1194/jlr.M051680.
28) Baker JR, Chaykin S. 1962. The biosynthesis oftrimethylamine-N-oxide. J Biol Chem 237:1309 –1313. http://dx.doi.org/10.1016/0006 -3002(60)90062-7.
29) Tang WHW, Wang Z, Kennedy DJ, Wu Y, Buffa JA, Agatisa-Boyle B, Li XS, Levison BS, Hazen SL. 2015. Gut microbiota-dependent trimethylamine N-oxide (TMAO) pathway contributes to both development of renal insufficiency and mortality risk in chronic kidney disease. Circ Res 116:448–455. http://dx.doi.org/10.1161/CIRCRESAHA.116.305360.
30) Bae S, Ulrich CM, Neuhouser ML, Malysheva O, Bailey LB, Xiao L, Brown EC, Cushing-Haugen KL, Zheng Y, Cheng T-YD, Miller JW, Green R, Lane DS, Beresford SAA, Caudill MA. 2014. Plasma choline metabolites and colorectal cancer risk in the women’s health initiative observational study. Cancer Res 74:7442–7452. http://dx.doi.org/10.1158/ 0008-5472.CAN-14-1835.
31) Trøseid M, Ueland T, Hov JR, Svardal A, Gregersen I, Dahl CP, Aakhus S, Gude E, Bjørndal B, Halvorsen B, Karlsen TH, Aukrust P, Gullestad L, Berge RK, Yndestad A. 1 December 2014. Microbiota-dependent metabolite trimethylamine-N-oxide is associated with disease severity and survival of patients with chronic heart failure. J Intern Med http:// dx.doi.org/10.1111/joim.12328.
32) Wang Z, Tang WHW, Buffa JA, Fu X, Britt EB, Koeth RA, Levison BS, Fan Y, Wu Y, Hazen SL. 2014. Prognostic value of choline and betaine depends on intestinal microbiota-generated metabolite trimethylamineN-oxide. Eur Heart J 35:904–910. http://dx.doi.org/10.1093/eurheartj/ ehu002.
33) Gao X, Liu X, Xu J, Xue C, Xue Y, Wang Y. 2014. Dietary trimethylamine N-oxide exacerbates impaired glucose tolerance in mice fed a high fat diet. J Biosci Bioeng 118:476 – 481. http://dx.doi.org/10.1016/ j.jbiosc.2014.03.001.